Seurat’s Origin Story
- Tom Burness
- Feb 22, 2021
- 6 min read
Updated: May 1, 2024
A Journey from Lab and Lasers to Product and Market
View this story on Medium
Seurat’s story begins in 2009. That winter, I attended a seminar at Stanford University on the National Ignition Facility (NIF), given by the facility’s Director, Ed Moses. The NIF is a part of the Department of Energy’s Lawrence Livermore National Laboratory and its mission is to conduct cutting-edge fusion energy research. This research could one day provide virtually unlimited power. The NIF houses 192 of the world’s most energetic lasers, which are all directed to a single small target. These lasers deliver over two million joules of energy to that target in about twenty billionths of a second — this is 1,000 times more power than the United States uses at any instant in time. At the time, I was wrapping up my master’s program at Stanford in mechanical engineering. Needless to say, I was captivated.
By June of that year, I had started a position at the NIF, working on a project to architect a fusion powerplant with the NIF blueprint as a baseline. The goal of the project was to make these massive lasers fire 16 times a second, creating the same nuclear fusion reaction that powers the sun. This is the same goal of fusion energy being pursued by several groups around the world.
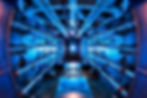
A view of a small part of the NIF laser system. The 192-laser system is the size of three football fields.
The promise of virtually unlimited power is compelling, but the technical challenges to achieve it are daunting. The chamber that houses this reaction needs to be able to withstand intense temperature swings and transfer the energy out of the chamber to make electricity. The space essentially needs to house a sun. As you can imagine, the stresses on the fusion chamber walls are brutal — every laser shot causes a multi-hundred-degree temperature fluctuation in the wall. These massive, rapid temperature fluctuations eventually cause cracks to form, leading to structural failure. Operating near 600C (1100F), there are only a handful of very specialized materials that can survive these conditions.
A material was finally found that met these extreme requirements — a very specialized steel that uses nanoparticles to give it high-temperature strength. Unfortunately, this material was unweldable. This was a big problem since the chamber needed to be twelve meters across. The walls themselves needed to be two meters thick, and with lots of internal structures, they could only be made by welding smaller sections together to make the whole structure. It became clear that the best way to manufacture these structures was through a type of Additive Manufacturing (AM or 3D Printing) called Laser Powder Bed Fusion (L-PBF).
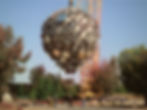
The target chamber for the National Ignition Facility being moved into place in 1999. The chamber design that I worked on was vastly more complex, needing to cope with 16 shots a second rather than just one per day. For a view of the chamber, see Star Trek “Into Darkness” (00:36.26, 01:40.41, or 01:44.15).
L-PBF had been demonstrated to be technically capable of processing the special type of steel and meeting the mechanical property requirements needed for such an intense environment. The problem with L-PBF however, is that it is extremely slow. The fastest available machine in the world, even today, would take nearly two hundred years to print just a single fusion chamber at the resolution needed.
This problem drove me, along with my co-inventors Bassem El-Dasher, Andy Bayramian, Joe Farmer, and Sharon Torres, to work to understand the fundamental barrier preventing Additive Manufacturing from scaling. Together, we figured out how to remove that barrier and devised a system that could scale to have a high enough throughput to manufacture these chambers in a reasonable timeframe.
In the L-PBF process, there is an inverse relationship between resolution and throughput — the higher the resolution, the lower the throughput. The process works by shining a laser onto a thin layer of metal powder. The intense laser spot melts the powder and welds it to the layer below. The melting speed is related to the laser power — a higher power laser melts material faster. The resolution of the metal (how fine of a detail can be printed) is related to the laser spot size — the bigger the spot size, the worse the resolution. The problem arises from the existence of an optimum power per unit area to create good quality material. To summarize, to print faster, laser power AND laser spot size must be increased, increasing print rate, but decreasing resolution. This fundamental limitation would not meet our needs for the fusion chamber, which meant we needed to try something different.
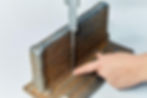
High deposition rate AM techniques can produce many kg of material per hour, but with vastly reduced resolution. The tradeoff between build rate and resolution is a fundamental limitation of scaling conventional metal AM.
The way that the L-PBF industry has attempted to overcome this barrier is to simply add more lasers. This method turns out to be not so simple. Back in 2015, the first companies were just starting to scale from one laser per machine to two. In 2021, the industry-standard is now four lasers. At the current rate of progress, we would need to wait two hundred years for L-PBF to scale to be fast enough to print the Livermore fusion chamber.
We needed to come up with a way to scale while maintaining resolution. We needed to break the coupling between resolution and print rate. What better way to accomplish this than to pattern the laser? What if every pixel could function as an individual laser? If possible, every pixel in the pattern would have the defining feature as its own independent beam — all with the optical simplicity of a single beamline.
The problem is, off-the-shelf solutions such as electronically driven liquid crystal displays or micro-mirror arrays are fraught with difficulty. Patterning a high-power laser is not easy. The device that transfers the pattern to the laser needs to be able to survive. It gets worse… If the goal is to print ever faster, ever higher power levels are required!
Our team searched for a solution. It turned out, the answer was already being developed at LLNL in parallel. The project, titled LEOPARD, created a device called an Optically Addressed Light Valve (OALV). The OALV allowed for the NIF laser beam to be patterned with high-resolution images that could be programmed to block or let light through each one of its “pixels”. Every pixel in the OALV defined its own laser spot, effectively creating a multitude of individual lasers out of one very powerful laser. By 2010 this project had won an R&D100 award and was installed at the NIF, facilitating fusion energy research.
Over the next 5 years, I worked to develop a >5kW laser system to incorporate a NIF OALV. This system demonstrated that we could project laser light down to a bed of metal powder, weld a patterned area in an instant, and build a multi-layer part using this technique. This system architecture was the solution, opening endless possibilities for Additive Manufacturing. With this technique, the ability to operate with millions of laser spots in parallel became possible. Printed feature resolution was no longer tied to the overall laser beam size. Instead, resolution and laser power could be separately engineered to satisfy the requirements of the system.
Finally, we decoupled resolution from throughput.
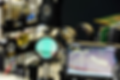
The Optically Addressed Light Valve is the key enabling technology that lets Seurat scale AM printing rates without losing resolution.
I met my soon-to-be co-founder, Erik Toomre, early on in 2015. We shared grand visions for what a future could hold with the advent of a scalable additive manufacturing process. After many late nights, on May 23rd, 2015, I proved this method; now called Area Printing, to be capable, and first demonstrated melting metal. Soon thereafter on July 2nd, Erik and I co-founded Seurat. By January 2016, we had secured the technology license from LLNL and began fundraising and commercializing the technology.
Seurat first raised a seed round from True Ventures in June 2016, followed by a Series A in the fall of 2017 also led by True Ventures. Seurat raised a Series B in June of 2021 led by Capricorn’s Technology Impact Fund (TIF II), and brought in additional interest into the round in the form of a Series B Extension also led by Capricorn’s TIF II. As of January 2022, Seurat has since raised a total of $79.5M, with key strategic investors from the automotive, energy, and manufacturing industries.
Seurat’s Area Printing technology has the capability to manufacture metal components more cost-effectively than not just other Additive Manufacturing techniques, but conventional techniques of machining, casting, and forging.
Seurat has an expert team and proven technology. In January 2021, we demonstrated printing stainless steel with mechanical properties that exceed industry standards, proving that we not only can print beautiful parts but can also create quality metal. In 2020, Seurat launched its first large-scale customer qualification program for the energy industry, and began qualifying nickel based alloy In718 and M300 tool steel. Seurat is now scaling to deliver the first production Area Printing machines, deployed in a state-of-the-art pilot factory in Wilmington, MA, bringing about a new age of manufacturing.
We are excited to share more information about how Area Printing works and the benefits it brings to metals manufacturing over the next months and years. We appreciate our partners, including LLNL, who have helped us get this far, and we look forward to working with many others over the coming years to change the way metal components are made. This technology is the future, and it will revolutionize manufacturing as we know it.